A PhRMA Working Group's advice on applying QbD to biotech.
By Taruna Arora, Roger Greene, Jennifer Mercer, Paul Tsang, Meg Casais, Stuart Feldman, Jutta Look, Tony Lubiniecki, Joseph Mezzatesta, Stefanie Pluschkell, Mark Rosolowsky, Anurag S. Rathore, PhD, Mark Schenerman, Tim Schofield, Samantha Sheridan, Paul Smock, Sally Anliker, Lois Atkins, Bernerd McGarvey, Bruce Meiklejohn, Jim Precup, John Towns
ABSTRACT
The International Conference on Harmonization (ICH) Q8(R2), Q9, and Q10 guidelines provide the foundation for implementing Quality by Design (QbD). Applying those concepts to the manufacture of biotech products, however, involves some nuances and complexities. Therefore, this paper offers guidance and interpretation for implementing QbD for biopharmaceuticals, from early-phase development steps such as identifying critical quality attributes and setting specifications, followed by the development of the design space and establishing the process control strategy; to later stages, including incorporating QbD into a regulatory filing and facilitating efficient commercial processes and manufacturing change flexibility post licensure.
Quality by Design (QbD) is a concept applied to the design and development of biopharmaceutical molecules and manufacturing that entails building quality into the process and product in a systematic, science- and risk-based manner. The QbD concepts outlined in the International Conference on Harmonization (ICH) Q8(R2), Q9, and Q10 guidelines provide the foundation for implementing QbD during specific stages of product development.1–3 A critical component of QbD is understanding the needs of the patient and the specific quality attributes of the product that are linked to safety and efficacy. Thus, to implement QbD, it is critical to have a fundamental understanding of the functional relationships between patient needs, product quality attributes, analytical capabilities, and the manufacturing process.
Pharmaceutical product sponsors begin by identifying product requirements to meet patient needs and then determine the quality target product profile (QTPP) and the critical quality attributes (CQAs) required to meet those patient needs. Based on this information, the sponsor then designs the molecule, the manufacturing process, and the control strategy to ensure that the desired product quality is met consistently.
The QbD approach can be maintained throughout the lifecycle of the product to facilitate innovation and continuous improvement based on expanded knowledge and new technologies. This knowledge is gained during development and grows with more manufacturing experience through process characterization, scale-up, technology transfer, and manufacture, as well as through increased patient exposure to the product. This approach encourages incorporating prior process and product knowledge and experience-based methodologies (i.e., platform knowledge) into manufacturing throughout the life of the product.
The QbD approach was introduced into the US Food and Drug Administration's chemistry, manufacturing, and controls (CMC) review process in 2004 with the aim of enhancing and modernizing the regulation of pharmaceutical manufacturing and product quality.4 The goal of QbD is to develop robust, well-understood processes that 1) deliver a product meeting the QTPP and 2) are controlled by defined steps within the manufacturing process and that allow for manufacturing process changes within an established design space of input variables and operating parameters without negatively affecting process attributes or identified CQAs. When a product is developed using a QbD approach, the impact of raw and starting materials and process parameters on product quality are well understood and the sources of process and product variability are well-known and controlled. In contrast, traditional pharmaceutical manufacturing relies heavily on end-product testing and the process typically lacks the flexibility needed to respond to variables encountered during manufacturing. The outcome is often "quality by conformance" and a rigid, static manufacturing operation that is more susceptible to multiple manufacturing changes post licensure, thus requiring continuous updating of the application through burdensome global regulatory supplements, variations, or amendments.
This paper focuses on the factors to consider when applying the QbD concepts outlined in ICH Q8(R2), Q9, and Q10 to biotechnology products. The paper is intended to capture and reflect both the current and future state of QbD implementation. Therefore, new tools and terminology (e.g., expanded change protocol and the post-approval management plan) have been included with an expectation that the paper will be revised in the future based on new information and experiences, such as the mock biotech common technical document (CTD) sections being developed by the European Federation of Pharmaceutical Industries and Associations (EFPIA) and the CMC Biotech Working Group (formerly Conformia), and the US FDA's CMC Biotech Pilot Program.
This position paper begins by addressing patient needs and product quality attributes, followed by a discussion of characterization development studies and the development of the design space and control strategies. It then addresses how to incorporate QbD into a regulatory filing and finally, how to facilitate efficient, cost-effective commercial processes and manufacturing change flexibility post licensure. Although biologic and biotechnology products often present a higher level of complexity than small molecules in terms of manufacturing process or product structure, the concepts of QbD are the same as those for small molecules. This paper describes the nuances and complexities involved in implementing QbD in the manufacture of biotech products and offers guidance and interpretation for doing so. The scope of this paper is limited to well-characterized protein products, in which the natural molecular heterogeneity, impurity profile, and potency can be defined with a high degree of confidence.
ESTABLISHING CRITICAL QUALITY ATTRIBUTES, SPECIFICATIONS, DESIGN SPACE, AND CONTROL STRATEGY FOR A BIOTECH PRODUCT
QbD is centered on the patient.Understanding the patient's needs for managing his or her disease state helps define the QTPP. Safety, efficacy, convenience, compliance, and cost effectiveness of the product must be considered. Next, CQAs of the product are identified, based on the understanding of the impact of various quality attributes on the safety and efficacy of the product. The initial assessment is based on molecular design and known attributes of the molecule. These include not only a comprehensive understanding of the molecular structure but also data collected from clinical and nonclinical studies that have been performed with the molecule and with other relevant molecules, as well as other applicable published knowledge. This process of assessing quality attributes and determining their criticality is ongoing, and is revisited continuously throughout the product lifecycle as more data become available. These data are also used to set appropriate specifications for the drug substance and the drug product, and to develop the overall control strategy.
|
The CQAs are also used to design the molecule and the manufacturing process to meet patient needs and endpoints for safety and efficacy, as well as to control process and product quality. Further, the process is optimized and characterized, and the cumulative data set is used to define the critical process parameters, process design space, and the process control strategy, which together ensure that appropriate quality is maintained and variability is managed during the manufacturing, storage, and distribution of the product. This approach is schematically illustrated in Figure 1. A more detailed discussion of these steps is presented below.
Applying QbD Early in Development
Products that are early in the development process are in a unique position to participate fully in prospective QbD during development. Consequently, companies may be able to develop an efficient, cost-effective commercial process for such products, and benefit from a more flexible reporting mechanism for changes to the application after approval. This situation is expected to provide an opportunity for manufacturing to incorporate new technology more quickly and easily and to make changes within the design space without regulatory agency review and approval.
Products in early stages of development usually have a limited amount of manufacturing history and limited commercial-scale experience. As commercial-scale manufacturing data are gathered post-approval, new knowledge can be continuously integrated into the pre-approval understanding. The resulting comprehensive knowledge can then be used to support process changes and the assessment of their potential impact on critical quality attributes based on all available lifecycle data.
Molecular Design and Engineering
For biotechnology products, a key element in implementing QbD is engineering the molecule itself. A number of strategies are currently used by investigators to alter the properties of the molecule to achieve the desired balance among efficacy, stability, safety (such as immunogenicity), and manufacturability. These strategies include engineering the primary sequence to incorporate chemical and post-translational modifications for improved stability; recombinant and chemical fusion approaches for improved half-life and potency; and affinity maturation by phage display or transgenic mouse (e.g., xenomouse, Humax, and velocity mouse) technologies. Understanding the structure and functional attributes of therapeutic proteins, including monoclonal antibodies (MAbs), is key to developing the design space, because that understanding facilitates the selection of desirable quality attributes during molecular design while ensuring that bioactivity of the protein therapeutic is maintained.
Technologies based on combinatorial libraries and transgenic mice can generate a diverse panel of antibodies and protein-based candidates selected for binding and specificity for a target. Further selection and screening may involve recombinant cloning from hybridomas, humanization, and fusions (e.g., Fc or human serum albumin, PEGylation) and the use of stringent parameters like in vitro and in vivo assessment of biological activity. Other key factors in the selection of an efficacious molecule include affinity and the rate of dissociation from the target. Protein engineering also can be applied to increase tissue penetration and distribution.
Switching the isotype of an antibody product to gain desired effector functions is one approach that has been practiced. In some cases, an IgG1 isotype is ideal for cell killing, whereas IgG2 is a favored isotype for eliminating the effector function of an antibody on target binding. For further enhancement of cell killing by an antibody, technologies focused on antibody Fc modifications by mutations or glycoengineering as well as fusions to toxin or drug conjugates have emerged and been taken to clinical trials for some targets.
Engineering the protein sequence is also used to reduce the risk of an immune response. Murine and chimeric antibodies used as human therapeutics frequently produce an immune response that can result in a reduction in or loss of activity and an unfavorable pharmokinetic (PK) profile requiring frequent administration of a drug. Humanization, transgenic mice, and phage display approaches make it possible to select antibody sequences bearing high homology to human germline sequences with the goal of reducing the risk of immunogenicity. Nevertheless, the risk of immune response to a fully human antibody and other self proteins necessitates screening therapeutic protein candidates for immunogenicity using in silico methods, and in vitro assays measuring T-cell responses. Sequence engineering also can be used to eliminate T-cell epitopes in the product.
Besides rational design for efficacy and safety, science-based hypothesis-driven approaches are used for selection and early engineering to achieve balance in the optimal physical and chemical properties of proteins with respect to product stability during and after manufacturing. A common observation during the development and commercialization of protein products is the susceptibility of certain amino acids (e.g., asparagine and methionine) to chemical modifications. Deamidation of asparagines and oxidation of methionines in regions important for activity can affect efficacy in some products. Sequence engineering also may be applied to minimize risks of chemical instability.
In summary, protein design and engineering are powerful tools for enhancing efficacy, modulating immunogenicity, altering proteolytic stability, introducing chemical modification sites, and improving expression. Rational design approaches make it possible to identify and engineer better protein therapeutic candidates right from the start, which combined with process optimization can pave the way for less frequent dosing, increased efficacy, decreased manufacturing costs, and an improved safety profile.5–10
Using Laboratory, Nonclinical, and Clinical Studies to Identify and Assess CQAs
An important step in the bioproduct development process is the identification of the CQAs of the product that drive the assessment of the ability of the bioprocess and bioproduct to deliver the intended performance of the product.11 The initial step toward identifying the CQAs is achieving a full understanding of the molecular structure and product variants. Next, all the molecular quality attributes and potential quality attributes are ranked using risk-based tools. The objective of this exercise is to determine which quality attributes may have the potential to affect the performance (safety and efficacy) of the product. A typical monoclonal antibody product may have dozens of such quality attributes and all are considered and assessed. They include but are not limited to product-related variants, process-related impurities, formulation parameters, and essential attributes such as appearance. Examples of process-related impurities include host cell DNA, host cell protein, adventitious agents, residual medium supplements such as protein hydrolysates, and components such as nucleases or residual Protein A from chromatography resins used in the purification process.
There are multiple approaches to assessing the criticality of quality attributes. One such approach is to focus on the severity and uncertainty of impact (to safety and efficacy), with the goal of process and product design and the control strategy to minimize the probability of occurrence to reduce overall risk. The ability to detect a quality attribute during product development is required to assess its occurrence, thus necessitating the development of methods suitable for this detection and quantitation. The need to detect a specific attribute can decrease as its severity and occurrence are eliminated or minimized to an acceptable level with product knowledge and process control. Other approaches do not incorporate an assessment of occurrence, because CQAs are not tied to the ability of the process to control an attribute.
Prior product knowledge plays a key role in the risk assessment and consists of the accumulated laboratory, nonclinical, and clinical experience for any specific product quality attribute. It also can include relevant data from similar molecules and data from literature references. With the increasing use of platforms in the development of biotech products and process, such as monoclonal antibody products, it is expected that investments in creating this knowledge can be capitalized by applying it to several products once the platform has been established.12–19 This combined knowledge provides a rationale for relating the attribute to product safety and efficacy. As mentioned above, the assessment of the severity of the impact (to safety or efficacy) results in a list of quality attributes in order of criticality. The list may evolve as more product and process knowledge are accumulated.
Process-related components may be handled by considering their potential safety risk. One approach to assessing this safety risk is to evaluate an impurity safety factor (ISF) with an appropriate target or lower limit. The ISF is the ratio of the impurity LD50 to the maximum amount of an impurity potentially present in the product dose:
ISF = LD50 ÷ Level in product dose
in which LD50 is the dose of an impurity that results in lethality in 50% of the animals tested, and the level in product dose refers to the maximum amount of an impurity that could potentially be present and co-administered in a dose of product. The ISF is a normalized measure of the relationship between the level of an impurity resulting in a quantifiable toxic effect and the potential exposure of a patient to an impurity in the product. In the absence of an assay to detect an impurity, a conservative assumption is that all of the impurity in the process co-purifies with the product, and no clearance is achieved by the purification process. In cases where a sufficiently sensitive assay is available, the actual level of an impurity in the product is measured. Impurities can be eliminated from further consideration at any step where the safety risk is determined to be minimal, with an eye toward any impurity being removed or eliminated to as low a level as possible. An alternative strategy for process-related impurities, such as DNA or host cell proteins, is to demonstrate multi-log removal by validation that is conducted in a fashion similar to the validation of viral reduction or removal, thus obviating the need for a specification.
The criticality of quality attributes may be explored in appropriately designed in vitro and in vivo studies. An example of the assessment of the affect of deamidation on the bioavailability, potency, and immunogenicity of a monoclonal antibody has been detailed elsewhere.15 A combination of tools may be used to establish correlations between quality attributes and preclinical or clinical performance. Examples include:
- analytical tools such as native isoelectric focusing (IEF), ion-exchange chromatography (IEC), and online mass spectrometry to quantify the level of deamidation
- preclinical pharmacokinetics studies with the native and deamidated molecules
- in vivo potency studies with the native and deamidated molecules
- preclinical immunogenicity studies using extended dosing with the deamidated molecule
- determining the deamidation rate that would occur in vivo and assessing its relevance compared to the circulating half-life of the molecule.
All or a combination of some of these studies may help qualify the criticality of an attribute such as deamidation.
Setting Drug Substance and Drug Product Specifications
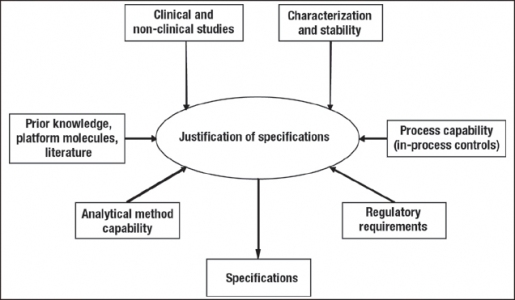
Product specifications can be proposed using a combination of approaches, consistent with the guidance in ICH Q6B (Figure 2).20 Where appropriate, certain attribute specifications are based on existing regulatory guidance. For other attributes, relevant limits or ranges are established based on several sources of information that link the attributes to the safety and efficacy of the product, similar to the way this information is used to assess the criticality of these attributes. These sources include but are not limited to:
- clinical experience with the product
- nonclinical studies with the product or the isolated variant or impurity such as binding assays, in vivo assays, and cell-based assays
- clinical and nonclinical studies with similar platform products
- published literature on other similar products, variants, or impurities
- the process control strategy and the extent of variability observed in the manufactured lots.
The difference between actual experience in the clinic and product specifications depends on our level of understanding of the impact that the attribute under consideration can have on the safety and efficacy of the product. This is illustrated in Figure 3 for two attributes, purity by IEC and host cell proteins. In the case of purity, the proposed specifications of >95% compared to the clinical experience of >98% would require either nonclinical studies relating the impurity or impurities to safety and efficacy, or clinical or nonclinical data on these species from another similar platform molecule or the literature.
The proposed specification for host cell proteins (<100 ng/mg) is significantly broader than the clinical experience (<10 ng/mg). This may be justified based on our understanding of the host cell proteins or data from other molecules within a platform, as it is recognized that the types of host cell proteins present can be as important as the level of host cell proteins.
In summary, identifying the CQAs and setting appropriate specifications and acceptable limits (or validating removal if applicable) are the foundational activities of implementing QbD for biologics. The former requires a risk assessment process to estimate the criticality of each quality attribute. Both activities depend heavily on prior knowledge from the sources listed above.
Developing the Design Space Bioprocess manufacturing can be conceptualized as a series of contiguous unit operations such that meeting the drug substance or drug product quality targets is an end result of a single or a combination of multiple unit operations. With such linkages between process steps and unit operations, it is possible to establish the design space for each unit operation. Per ICH Q8(R2), the design space is
the multidimensional combination and interaction of input variables (e.g., material attributes) and process parameters that have been demonstrated to provide assurance of quality. 1
Thus, the design space for a unit operation or process step encompasses the acceptable ranges for the critical process parameters of this step that will deliver product of the desired quality.
A thorough understanding of the factors that could affect the process is required. A risk assessment should be performed to identify the process parameters that should be characterized using small-scale models and tools such as design of experiments (DoE). One approach commonly used to facilitate a cause-and-effect analysis is the "fishbone" or derivative/one branch Ishikawa diagram (Figure 4). Such a diagram provides a graphical representation of the relationship between process operating parameters and unit operation outcomes. For the reactor expansion unit operation, for example, the cell growth, viable cell density, culture viability, and glucose concentration may be affected by the inoculum, medium, bioreactor operation, and harvest. Scientific understanding and prior knowledge can be used together with quality risk management to prioritize which process parameters to study. One tool that is useful for documenting factor risks is failure mode effects analysis (FMEA), which uses the severity, probability, and detectability of each factor to rank risks within a process step, and thereby select factors for subsequent study.2 The accumulated information from similar molecules or experiences with similar technologies may be used to identify variables and define an initial design space for the process step of a new molecular entity.
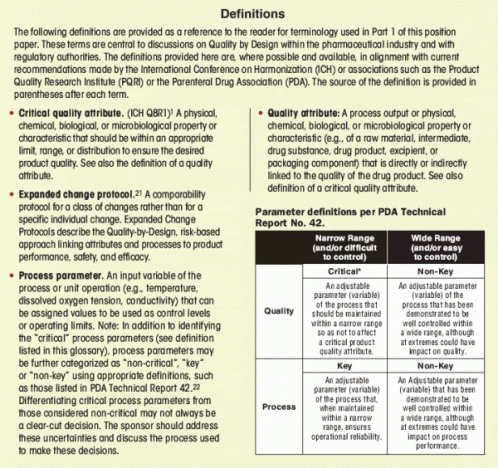
Whereas operating ranges of a process in early development are primarily based on experience with equipment and platform technologies, design space limits will become available during late development and finally established during process characterization using small-scale models and tools like multivariable DOE. (For a description of process characterization, see PDA Technical Report No. 42). The refinement of the design space is based on the QTPP and the knowledge gained throughout development. As knowledge increases, some of the potentially critical and key process parameters will be identified as noncritical or non-key so that the number of critical and key process parameters may be reduced during late development.
PARTS 2 AND 3 OF THIS ARTICLE
Parts 2 and 3 of this article appeared in the December 2009 and January 2010 issues of BioPharm International. Part 2 covers the use of DOE to define the design space, unique considerations for process development for biopharmaceuticals, the establishment of a control strategy, and the placement of QbD information in a regultory application. Part 3 discusses continuous verification and post-approval changes, including topics such as verification at large scale, refinement of the design space, process changes and comparability, comparability protocols and expanded change protocols, marketed products, and a CMC post-approval management plan. It also includes the overall conclusions.
ABOUT THE AUTHORS
Taruna Arora is a principal scientist, protein science, Roger Greene is the director of regulatory affairs, Jennifer Mercer is the director of regulatory affairs, CMC, and Paul Tsang is the executive director of quality, all at Amgen Inc.; Meg Casais is the director of global regulatory affairs, CMC, and Stuart Feldman is the director/CMC, global regulatory affairs, both at Schering Plough; Jutta Look is the senior manager, CMC regulatory affairs at Novartis Pharma AG; Tony Lubiniecki is the vice president of biopharmaceutical development & marketed product support at Centocor R&D, Inc.; Joseph Mezzatesta is the assistant director of regulatory CMC, corporate regulatory affairs, at Sanofi-Aventis; Stefanie Pluschkell is an associate research fellow in global CMC, biologics and devices, at Pfizer Inc.; Mark Rosolowsky is the executive director of global regulatory sciences–CMC at Bristol Myers Squibb; Anurag Rathore is a biotech CMC consultant and faculty member at the Indian Institute of Technology, Delhi, India; Mark Schenerman is the vice president of analytical biochemistry at MedImmune; Tim Schofield is the director of US regulatory affairs at GlaxoSmithKline; Samantha Sheridan is the director of regulatory affairs at Shire Pharmaceuticals; Paul Smock is a director and quality product leader in Wyeth Biotech, Wyeth Pharmaceuticals; Sally Anliker is the director of regulatory affairs CMC, Lois Atkins is a principal consultant, CMC regulatory affairs, Bernerd McGarvey is an engineering advisor in the process engineering center, Bruce Meiklejohn is a principal fellow, regulatory COE-biotech, Jim Precup is a research scientist in manufacturing, science and technology, and John Towns is the senior director of global CMC regulatory affairs, all at Eli Lilly and Company. Towns is also the chair of the working group, 317.276.4079, [email protected]
REFERENCES
1. International Conference on Harmonization. Q8(R2), Pharmaceutical development. Geneva, Switzerland. 2009 Aug.
2. US Food and Drug Administration. Guidance for industry: Q9 Quality risk management. Bethesda, MD; 2006.
3. US FDA. Guidance for Industry: Q10 Quality systems approach to pharmaceutical CGMP regulations. Bethesda, MD; 2006.
4. US FDA. Guidance for industry: PAT—A framework for innovative pharmaceutical development, manufacturing, and quality assurance. 2004 Sept.
5. Lonesberg N. Human antibodies from transgenic animals. Nature Biotech. 2005;23:1:117–25.
6. Perry LC, Jones TD, Baker MP. New approaches to prediction of immune responses to therapeutic proteins during preclinical development. Drugs R D. 2008;99(6):385–96.
7. Beckman RA, Weiner LM, Davis HM. Antibody constructs in cancer therapy; protein engineering strategies to improve exposure in solid tumors. Cancer. 2007;109(2):170–9.
8. Ravetch J. Antibodies therapeutics:isotype and glycoform selection. Expert opin biol ther. 2007; 7(9):1401–13.
9. Rehder DS, Chelius D, McAuley A, Dillon TM, Xiao G, Crouse-Zeineddini J, et al. Isomerization of a single aspartyl residue of anti-epidermal growth factor receptor immunoglobulin gamm2 antibody highlights the role avidity plays in antibody activity. Biochemistry. 2008;47(8):2,518–30.
10. Honegger A. Engineering antibodies for stability and efficient folding. Handb Exp Pharmacol. 2008;181:47–68.
11. Rathore AR, Winkle H. Quality by Design for pharmaceuticals: Regulatory perspective and approach. Nature Biotechnol. 2009. 27(1):26–34.
12. Barrett YC, Ebling W, Pieniaszek H, Billheimer J, Seiffert D. Validation and implementation of drug-dependent antibody assays in clinical trials for safety monitoring of patients dosed with roxifiban, an orally bioavailable glycoprotein IIb/IIIa antagonist. J Pharm Biomed Anal. 2007 Aug 15;44(4):938–46.
13. Klakamp SL, Lu H, Tabrizi M, Funelas C, Roskos LK, Coleman D. Application of analytical detection concepts to immunogenicity testing. Anal Chem. 2007 Nov 1;79(21):8,176–84.
14. Mire-Sluis AR, Barrett YC, Devanarayan V, Koren E, Liu H, Maia M, et al. Recommendations for the design and optimization of immunoassays used in the detection of host antibodies against biotechnology products. J Immunol Methods. 2004 Jun;289(1–2):1–16.
15. Schenerman MA, Axley MJ, Oliver CN, Ram K, Wasserman GF. Using a risk assessment process to determine criticality of product quality attributes. In: Rathore AS, Mhatre R, editors. Quality by design for biopharmaceuticals: principles and case studies. Hoboken: Wiley; 2009.
16. Gupta S, Indelicato SR, Jethwa V, Kawabata T, Kelley M, Mire-Sluis AR, et al. Recommendations for the design, optimization, and qualification of cell-based assays used for the detection of neutralizing antibody responses elicited to biological therapeutics. J Immunol Methods. 2007 Apr 10;321(1–2):1–18.
17. Rathore A, Branning R, Cecchini D. Design space for biotech products. BioPharm Int. 2007 April;20(4):36–40.
18. Harms J, Wang X, Kim T, Yang J, Rathore AS. Defining design space for biotech products: Case study of Pichia pastoris fermentation. Biotechnol Prog. 2008:24(3):655–62.
19. Godavarti R, Petrone J, Robinson J, Wright R, Kelley BD. Scale-down models for purification processes: approaches and applications. In Rathore AS, Sofer G, editors. Process validation in manufacturing of biopharmaceuticals. New York: Taylor and Francis; 2005.
20. US FDA. Guidance for industry: Q6B specifications: Test procedures and acceptance criteria for biotechnological/biological products. Rockville, MD; 1999 Aug.
21. Submission of Quality Information for Biotechnology Products in the Office of Biotechnology Products; Notice of Pilot Program. Federal Register. July 2 2008;73(128):37972–4.
22. Parenteral Drug Association. PDA Technical Report 42. Process Validation of Protein Manufacturing. Bethesda, MD: PDA; Sept 2005;59(S-4):1–28.
Note: This article was originally published in November 2009 in BioPharm International Volume 22, Issue 11, and can be found online here. See part 2 here and part 3 here.